Research in the field of mitochondrial diseases has made great progress in the last 15 years. While for a long time scientists worked on understanding the causes and biological consequences of mitochondria and cells with mutations, the focus is now much more on therapeutic methods and clinical trials
Two illustrative examples of active research:
Reparing what's broken
Using old drugs in a new and personalized way
Repairing what's broken
The majority of mitochondrial diseases are caused by alteration of a single or few base pairs of 'letters' of our DNA code. A small change in the 3.2 billion base pairs of a human being. If it were possible to correct this single letter - so called 'gene editing' - the disease could be cured or at least alleviated.
The part of DNA that contains the blueprint for mitochondria is the only part of DNA that exists in two places. The vast majority of genes are located in the nucleus of each cell - where the overwhelming majority of DNA is located ('nuclear DNA'). But a very small portion of DNA - 37 genes in all - is located in the mitochondria themselves ('mitochondrial DNA'). Depending on where a DNA base is mutated (in the nucleus versus in the mitochondrion itself) and needs to be corrected by gene editing, different technologies may need to be used to 'repair' the mutation.
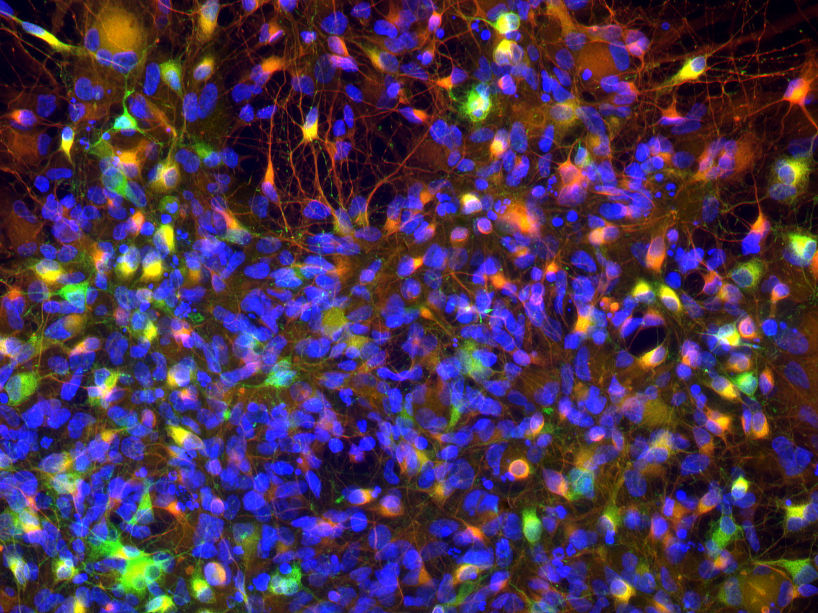
For nuclear DNA, techniques that can replace parts of the genome have existed for many years. The technology that might be crucial for a breakthrough in this area, was discovered in 2012 and the two discoverers were awarded the Nobel Prize in 2020. CRISPR/Cas9 technology makes it possible to identify a piece of DNA, cut it out and replace it with a new piece of DNA - all in a simple process and with high precision.
On the one hand, this enables a better understanding of mitochondrial diseases through better test tube ('in vitro') models or better animal models. On the other hand, this technology also offers the potential to make changes in a living human ('in vivo') to significantly improve or cure the disease ('Gene Therapy'). The genetic 'tools' required for this are typically introduced into the cells to be repaired via viruses.
However, the risk of such a repair in living humans is of course very high. For example, it is essential to prevent the alteration of additional sites (so called "off-target effects"), which could lead to irreversible destruction of other important parts of DNA. A first gene therapy in humans was performed with CRISPR in a clinical trial in 2020. In parallel, several clinical trials are underway with other gene therapy tools.
For mitochondrial DNA, until recently, there was no approach that could make gene therapy feasible in the future, as the CRISPR/Cas9 technology cannot be inserted into mitochondrial DNA, which is surrounded by two membranes. A potential breakthrough, however, was made in 2020 when an enzyme was discovered that can precisely alter individual base pairs of mitochondrial DNA without having to cut the DNA to do so. In 2021 this technology, with the complex name "double-stranded DNA deaminase (DddA)-derived cytosine base editor (DdCBE)“, was shown for the first time to alter base pairs of mitochondrial DNA quite precisely in living mice.
Another potential alternative for treating mitochondrial DNA defects is to provide a healthy copy of a mitochondrial gene that will relocate into the nucleus (so called "allotopic expression"). This approach is currently being tested for the mitochondrial disease LHON (Leber hereditary optic neuropathy), which causes loss of vision. The ongoing clinical trials appear to show positive results after injection of the gene therapy vectors in the eyes of the patients.
While the road to safe gene therapy for many mitochondrial diseases with complex manifestations in several different organs is still long, these technologies, all developed within the last decade, can lead to major research successes. These advances also depend on the development of better cellular and animal models in a timely manner, as both mechanisms of action and therapeutic approaches can be better explored through these models.
Using old drugs in a new and personalized way
For mitochondrial diseases, a number of drugs are on their way to approval in clinical trials. However, approval processes for new drugs take a very long time. Complicating matters for mitochondrial diseases is the fact that there are so many different subtypes, each with only a rather small number of patients. This makes clinical trials with large numbers of participants de facto impossible.
A much faster way to help patients could be to use existing drugs that have already been approved for different indications. Since these drugs have already been approved for a certain condition, their safety has been extensively tested on large numbers of individuals. If some of these drugs show efficacy against certain mitochondrial diseases, they could be approved for this new indication in a simplified approval process known as 'drug repurposing'. For rare diseases, like mitochondrial diseases, this process can be further promoted to pharma industry by giving certain incentives once the drug to be repurposed can acquire a certain status (the so called 'orphan drug designation'). Overall, the process of drug repurposing would lead to much faster treatment options for these patients.
The question is therefore: How do we find already approved drugs that also help against mitochondrial diseases, especially since there are many subtypes of these diseases?
A very personalized approach is to take cells from diseased individuals. In these cells, the patient-specific cellular defects can be analyzed. A large collection of drugs is then tested on these cells to see if they improve these cellular defects.
Large drug databases already exist with several thousand drugs that can be used to perform these analyses on real cells from patients using high-throughput laboratory robotics.
Medicine has also made great progress with the cells themselves in the last 15 years. A major problem was that cells with a high energy demand, such as brain or heart cells, can be more strongly affected by mitochondrial diseases. However, it is very difficult to gently remove these cells from patients - especially children.
This problem was solved by a medical invention that was recognized by the Nobel Prize in 2012: the 'induced pluripotent stem cells (iPSC)'. In the development of a human, all cells start from a starting point, the 'embryonic stem cells', which then 'differentiates' into different cell types. The use of embryonic stem cells for research is however challenging and controversial in some countries. What scientists were able to show in 2006 was that you can take another cell from a human being, e.g. a skin cell, and 'reprogram' it to a state similar to an embryonic stem cells simply by increasing the expression of certain genes. With this method, one can, for example, reprogram a skin cell back into a iPSC cell, to then differentiate it into a brain or heart cell by adding other proteins again. In this way, after a simple skin biopsy of a patient, one can 'produce' a cell model that is able to generate brain or heart cells that contain the same nuclear and mitochondrial DNA of the original patient. These differentiated cells can then be tested against a drug database in the method described before.
Already in 2017, this approach was piloted for a group of mitochondrial diseases. In the pilot study, a specific drug was identified from a group of several hundred drugs as a potential treatment candidate. The drug is now being tested for its efficacy in this disease in a first trial with a cohort of patients. The initial results were very positive.
In the meantime, a European research project is underway to further investigate this approach with the largest publicly available drug database and further cell analysis technologies on this group of mitochondrial diseases in order to identify further drugs, possibly with even better efficacy.
The way forward
The two research directions presented here are just examples of a large number of different approaches being researched worldwide. They also show the great progress that has been made in medicine over the last 15 years, which has made therapeutic and curative options possible in the first place. Research funding is a key factor in determining how quickly progress can be made.
Better funding of this research can make a huge difference on many levels. It funds the researchers themselves, the expensive lab equipment, expensive clincial trial applications, improved use of patient registries, and the like.
The core mission of the MitoHelp Foundation is to fund this research.